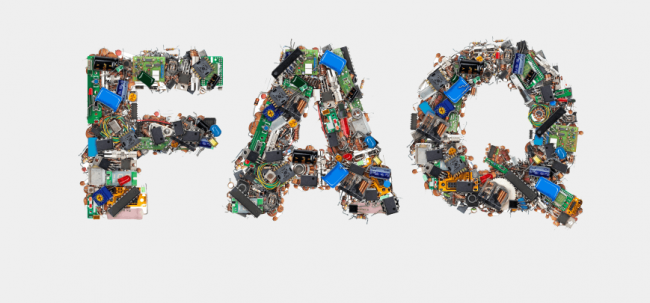
What components are included in the information network system in the EPC system structure?
In the context of an Electronic Product Code (EPC) system structure, the information network system plays a crucial role in managing and processing the data related to EPCs, RFID (Radio Frequency Identification) tags, and the associated supply chain information. The information network system encompasses various components that work together to enable the collection, storage, processing, and dissemination of EPC-related data. Some of the key components included in the information network system of an EPC system structure are as follows:
- EPCIS (Electronic Product Code Information Services): EPCIS is a core component of the information network system that provides a standardized interface for capturing, sharing, and querying EPC-related information. It allows different participants in the supply chain to exchange data about the movement and status of products, enabling end-to-end traceability.
- RFID Readers: RFID readers are responsible for reading the EPC data from RFID tags attached to products or items. They serve as the interface between the physical world and the information network system, enabling the capture of real-time data.
- RFID Tags: RFID tags are physical devices attached to products or assets, containing unique identifiers (EPCs) that can be read by RFID readers. These tags enable automatic identification and tracking of items throughout the supply chain.
- Middleware: Middleware serves as a bridge between RFID readers and the EPCIS, facilitating data filtering, processing, and integration. It ensures that the data captured by RFID readers is properly formatted and transmitted to the EPCIS for storage and further analysis.
- Database Management System (DBMS): The DBMS is used to store and manage the vast amount of EPC-related data collected from RFID tags. It provides efficient data storage and retrieval capabilities for the EPCIS.
- Event Handlers and Filters: Event handlers and filters are software components that process and analyze the raw RFID data to identify relevant events or changes in the supply chain. They help in generating meaningful and actionable information from the collected data.
- Data Analytics Tools: Data analytics tools are employed to analyze the collected data for valuable insights and patterns. They help in identifying trends, optimizing supply chain processes, and making informed business decisions.
- Security and Authentication Mechanisms: The information network system incorporates security measures to protect the integrity and confidentiality of the EPC-related data. Authentication mechanisms ensure that only authorized users can access and modify the information.
- Interfaces and APIs: Interfaces and Application Programming Interfaces (APIs) allow external systems or applications to interact with the information network system, facilitating integration with other enterprise systems, such as ERP (Enterprise Resource Planning) or WMS (Warehouse Management System).
- Data Integration and Exchange Protocols: To enable seamless data exchange and integration across different stakeholders, the information network system may use standardized data exchange protocols, such as Electronic Data Interchange (EDI) or Web Services.
The information network system in an EPC system structure forms the backbone of data management and communication, enabling the efficient tracking and tracing of products throughout the supply chain, enhancing visibility, and improving supply chain efficiency and responsiveness.
What are the obvious development trends in the power management product market?
The power supply voltage of the 1 processor is declining. The current buck converter has been unable to provide a high-efficiency power supply for a new generation of processors. A power supply system with synchronous rectification has emerged and became popular.3 Because digital power management technology can extend battery life and help improve the energy efficiency of the system, more and more portable electronic products and even power-hungry systems (such as data centers and wireless base stations) use digital power.Management technology.
The basic structure, main parameters and ideal characteristics of the amplifier?
An amplifier is an electronic device that increases the amplitude (or power) of an input signal to produce a larger output signal. The basic structure of an amplifier typically consists of the following main components:
- Input Stage: The input stage is the initial part of the amplifier circuit where the input signal is applied. It may include coupling capacitors, impedance matching components, and biasing networks to prepare the signal for amplification.
- Amplification Stage: The amplification stage is the core of the amplifier, where the input signal undergoes amplification. It often consists of active components such as transistors or operational amplifiers (op-amps) that provide the gain to increase the signal amplitude.
- Output Stage: The output stage is the final part of the amplifier circuit where the amplified signal is delivered to the load (e.g., speaker, motor, etc.). Output stages may include impedance matching and filtering components to optimize the transfer of power to the load.
Main Parameters of an Amplifier:
- Gain (Voltage Gain or Power Gain): Gain is a fundamental parameter of an amplifier and represents the ratio of the output signal amplitude to the input signal amplitude. It is typically expressed in decibels (dB) or as a unitless voltage or power ratio.
- Bandwidth: The bandwidth of an amplifier defines the range of frequencies over which the amplifier can provide substantial amplification without significant attenuation. It is usually specified as the frequency range within which the gain remains relatively constant.
- Input and Output Impedance: The input impedance represents the resistance seen by the signal source at the amplifier’s input, while the output impedance is the resistance seen by the load at the amplifier’s output. Impedance matching is essential for efficient power transfer.
- Linearity: The linearity of an amplifier refers to how faithfully it reproduces the shape of the input signal in the output signal. A linear amplifier produces an output proportional to the input, whereas a nonlinear amplifier introduces distortion.
- Noise Figure: Noise figure measures the noise added by the amplifier to the input signal. Low noise figure is desirable in applications where signal fidelity is crucial, such as communication systems.
- Distortion: Distortion measures the level of unwanted changes introduced to the output signal compared to the input signal. Low distortion is essential for high-fidelity audio and precise signal processing.
Ideal Characteristics of an Amplifier:
- Infinite Gain: In an ideal amplifier, the gain would be infinite, meaning it could amplify the input signal without any limitation.
- Infinite Bandwidth: An ideal amplifier would have an infinite bandwidth, allowing it to amplify signals of any frequency.
- Zero Noise: An ideal amplifier would introduce no noise to the input signal, preserving the signal’s quality.
- Zero Distortion: In an ideal amplifier, there would be no distortion, and the output signal would be an exact replica of the input signal.
- Infinite Input and Output Impedance: The input impedance would be infinite, ensuring no loading effect on the input signal, and the output impedance would be zero, providing an ideal power transfer to the load.
- Infinite Dynamic Range: An ideal amplifier would handle any input signal amplitude without saturation or clipping, offering an infinite dynamic range.
It’s important to note that no practical amplifier can meet all these ideal characteristics. The design of real-world amplifiers involves trade-offs to optimize performance for specific applications while considering factors like cost, power consumption, and size.
What is a heart rate control point?
The heart rate control point feature enables the collector to write the value of the heart rate control point to a heart rate sensor that supports the feature.
What is the inductive reactance? What is the calculation formula for the inductive reactance?
Inductive reactance is a property of inductors, which are passive electronic components that store energy in the form of a magnetic field when current flows through them. Inductive reactance is a measure of the opposition that an inductor presents to alternating current (AC). It is analogous to resistance in direct current (DC) circuits but specifically applies to AC circuits.
The inductive reactance (XL) is directly proportional to the frequency (f) of the AC signal and the inductance (L) of the inductor. The formula to calculate the inductive reactance is:
XL = 2πfL
Where: XL = Inductive reactance (in ohms, Ω) π (pi) ≈ 3.14159 (a mathematical constant) f = Frequency of the AC signal (in hertz, Hz) L = Inductance of the inductor (in henrys, H)
The inductive reactance is directly proportional to both the frequency and the inductance. As the frequency or inductance increases, the inductive reactance also increases. Inductive reactance is an essential parameter in AC circuit analysis, as it influences the behavior of inductors in the presence of alternating current.
Inductive reactance, like resistance, affects the magnitude of the current flowing through the inductor in an AC circuit. When an AC voltage is applied to an inductor, the inductive reactance opposes the flow of current, leading to a phase shift between the voltage and current. The magnitude of the inductive reactance determines the amplitude of this phase shift. As the frequency increases, the inductive reactance becomes more significant, leading to greater opposition to current flow in the inductor. Conversely, at very low frequencies (near DC), the inductive reactance approaches zero, making the inductor behave like a short circuit for DC signals.
What are the radio frequency identification systems classified according to the data volume of electronic tags?
Radio Frequency Identification (RFID) systems can be classified according to the data volume or storage capacity of electronic tags into the following categories:
- Low-Frequency (LF) RFID Systems: LF RFID systems typically operate in the frequency range of 125 kHz to 134 kHz. The data volume or storage capacity of electronic tags in LF RFID is relatively small. These tags are often used for simple identification purposes, such as access control, animal tracking, or proximity applications.
- High-Frequency (HF) RFID Systems: HF RFID systems operate in the frequency range of 13.56 MHz. HF RFID tags offer a higher data volume compared to LF RFID tags. They are commonly used in applications that require more data storage, such as contactless smart cards, public transportation cards, and electronic toll collection systems.
- Ultrahigh-Frequency (UHF) RFID Systems: UHF RFID systems operate in the frequency range of 860 MHz to 960 MHz. UHF RFID tags offer a larger data volume and faster data transfer rates compared to LF and HF RFID tags. They are widely used in logistics, inventory management, supply chain tracking, and asset tracking applications.
- Microwave Frequency RFID Systems: Microwave frequency RFID systems operate at higher frequencies above 1 GHz. These systems are less common compared to LF, HF, and UHF RFID systems and are typically used in specialized applications that require even larger data storage capacities.
The classification based on the data volume of electronic tags is essential in selecting the appropriate RFID technology for specific applications. Applications that require only simple identification and tracking may utilize LF or HF RFID systems, while those that demand more data storage and faster data transfer rates may opt for UHF RFID systems. Microwave frequency RFID systems are less common and typically used in niche applications that require even higher data storage capabilities.
What is the principle of electromagnetic law?
The principle of electromagnetic law is a fundamental concept in physics that describes the relationship between electric fields, magnetic fields, and electric charges. It is based on the discoveries of two prominent scientists: Michael Faraday and James Clerk Maxwell. The principle of electromagnetic law is summarized by Maxwell’s equations, which form the foundation of classical electromagnetism. These equations describe how electric and magnetic fields are generated and how they interact with electric charges.
The key principles of electromagnetic law can be summarized as follows:
- Faraday’s Law of Electromagnetic Induction: Faraday’s law states that a changing magnetic field induces an electromotive force (EMF) or voltage in a conducting loop. This phenomenon is the basis for generating electricity in electric generators and transformers. Faraday’s law explains the conversion of mechanical energy into electrical energy and vice versa.
- Ampere’s Circuital Law: Ampere’s law relates the magnetic field around a closed loop to the electric current passing through the loop. It states that the magnetic field is proportional to the current passing through the loop and inversely proportional to the distance from the current-carrying conductor. Ampere’s law is crucial in understanding the magnetic fields generated by electric currents.
- Gauss’s Law for Electricity: Gauss’s law for electricity relates the electric field to the electric charge distribution. It states that the total electric flux through a closed surface is proportional to the total charge enclosed by the surface. Gauss’s law helps to understand how electric charges create electric fields.
- Gauss’s Law for Magnetism: Gauss’s law for magnetism states that there are no magnetic monopoles, which means that magnetic field lines always form closed loops. Unlike electric fields, which originate from positive charges and terminate at negative charges, magnetic field lines always form continuous loops.
- Maxwell’s Displacement Current: One of Maxwell’s contributions to electromagnetic theory was the concept of displacement current. He realized that a changing electric field can produce an additional magnetic field, similar to how a changing magnetic field produces an electric field in Faraday’s law. This displacement current is significant in the propagation of electromagnetic waves.
By combining Faraday’s law, Ampere’s law, Gauss’s laws for electricity and magnetism, and the concept of displacement current, Maxwell’s equations mathematically describe the generation and interaction of electric and magnetic fields in space. These equations unify electricity and magnetism into a single electromagnetic theory and have profound implications for the understanding of light, radio waves, and all forms of electromagnetic radiation. Maxwell’s equations are essential in the development of modern technology, including communication systems, electronics, and electromechanical devices.
What is a capacitor?
A capacitor is a passive electronic component that stores electrical energy in an electric field. It consists of two conductive plates separated by an insulating material called a dielectric. When a voltage is applied across the plates, an electric field forms in the dielectric, causing the plates to store electric charge. The amount of charge stored in the capacitor is directly proportional to the applied voltage and the capacitance of the capacitor.
The capacitance (C) of a capacitor is a measure of its ability to store charge and is defined as the ratio of the amount of charge (Q) stored on one plate to the potential difference (V) between the plates:
C = Q / V
Where: C = Capacitance (in farads, F) Q = Charge stored on one plate (in coulombs, C) V = Potential difference between the plates (in volts, V)
The basic construction of a capacitor consists of two parallel conductive plates made of materials like aluminum, tantalum, or ceramic, with a dielectric material (insulator) placed between them. Common dielectric materials used in capacitors include air, paper, plastic films, ceramic, and electrolytic substances.
Capacitors are widely used in electronic circuits for various purposes, including energy storage, smoothing out voltage fluctuations (filtering), coupling and decoupling signals, and timing applications. They are essential components in power supplies, filters, oscillators, and many other electronic devices.
Capacitors come in various shapes and sizes, from small surface-mount capacitors used in compact electronic devices to large electrolytic capacitors used in power electronics applications. Each type of capacitor has specific characteristics, such as capacitance value, voltage rating, temperature tolerance, and frequency response, making them suitable for different circuit requirements.
What are the approximate aspects of PLC usage?
The usage of Programmable Logic Controllers (PLCs) is widespread across various industries and applications due to their versatility and ability to automate and control complex processes. PLCs offer numerous benefits and find applications in diverse fields. Some of the approximate aspects of PLC usage include:
- Industrial Automation: PLCs are extensively used in industrial automation to control and monitor manufacturing processes, assembly lines, and machinery. They can handle multiple inputs and outputs, making them ideal for managing complex automation tasks.
- Process Control: PLCs are employed in process control applications to regulate and optimize variables such as temperature, pressure, flow rate, and level in industrial processes like chemical manufacturing, oil refining, and water treatment.
- Motor Control: PLCs are widely used for motor control in various applications, including conveyor systems, pumps, fans, and robotic arms. They provide precise control over motor speed, direction, and acceleration.
- Home Automation: PLCs are used in smart home systems to control lighting, heating, ventilation, air conditioning (HVAC), security systems, and other household appliances, offering increased convenience and energy efficiency.
- Building Automation: In commercial buildings, PLCs are employed for building automation, managing tasks such as lighting control, access control, HVAC, and fire safety systems.
- Data Acquisition and Monitoring: PLCs can collect data from sensors and other devices to monitor and analyze process parameters, enabling predictive maintenance and improving system performance.
- Safety Systems: PLCs are used in safety-critical applications, such as emergency shutdown systems in industrial plants, where they can rapidly react to hazardous conditions and prevent accidents.
- Batch Processing: PLCs are commonly used in batch processing industries, such as pharmaceuticals and food and beverage manufacturing, to automate complex sequential processes.
- Remote Monitoring and Control: PLCs equipped with communication modules allow for remote monitoring and control of processes, enabling operators to manage systems from a central location.
- Training and Education: PLCs are utilized in educational institutions for teaching automation and control principles, providing hands-on experience in programming and troubleshooting industrial systems.
- Energy Management: PLCs are used in energy management systems to optimize energy consumption and improve energy efficiency in industrial and commercial facilities.
- Material Handling: PLCs are employed in material handling systems, such as conveyor belts and automated warehouses, for efficient movement and sorting of goods.
The above aspects represent a few of the many applications and areas where PLCs are utilized. The flexibility, reliability, and programmability of PLCs make them an indispensable tool for automation and control across a wide range of industries and processes.
What is a mixer?
In electronics and signal processing, a mixer is a device or circuit that combines two or more input signals to produce an output signal that contains the sum and difference frequencies of the input signals. It is a fundamental component used in various applications, including radio communication, audio processing, frequency conversion, and modulation/demodulation.
The primary purpose of a mixer is to shift the frequency spectrum of one signal to a different frequency range. This process is known as frequency mixing or frequency conversion. The mixer achieves this by using non-linear elements, such as diodes or transistors, which introduce nonlinearities into the circuit, resulting in the generation of sum and difference frequencies.
The basic concept of frequency mixing in a mixer can be described as follows:
Suppose we have two input signals:
- Signal 1 with frequency f1
- Signal 2 with frequency f2
When these two signals are applied to a mixer, the output will contain three main components:
- The sum frequency (f_sum) = f1 + f2
- The difference frequency (f_diff) = |f1 – f2|
- The original frequencies (f1 and f2)
Depending on the type of mixer circuit and its configuration, the original frequencies (f1 and f2) may or may not appear in the output.
Mixer circuits are used in various applications:
- Radio Frequency (RF) and Intermediate Frequency (IF) Stages: In radio receivers, mixers are used to convert incoming RF signals to a lower IF frequency for further processing and demodulation.
- Frequency Upconversion and Downconversion: In communication systems, mixers are used to shift the frequency of signals for transmission (upconversion) or reception (downconversion).
- Modulation and Demodulation: In communication systems, mixers are essential for modulating the carrier signal with the information signal and demodulating the modulated signal to extract the original information.
- Audio Signal Processing: In audio applications, mixers are used to combine audio signals from different sources, such as microphones or musical instruments.
- Signal Analysis: Mixers are used in signal analyzers to shift the frequency spectrum of the input signal for specific analysis purposes.
- Frequency Synthesizers: Mixers are used in frequency synthesizers to generate precise and stable output frequencies by combining signals from different sources.
Overall, mixers play a crucial role in various electronic systems by allowing the manipulation of frequency and facilitating the integration of multiple signals for processing and communication purposes.
COMMENTS